النبات
مواضيع عامة في علم النبات
الجذور - السيقان - الأوراق
النباتات الوعائية واللاوعائية
البذور (مغطاة البذور - عاريات البذور)
الطحالب
النباتات الطبية
الحيوان
مواضيع عامة في علم الحيوان
علم التشريح
التنوع الإحيائي
البايلوجيا الخلوية
الأحياء المجهرية
البكتيريا
الفطريات
الطفيليات
الفايروسات
علم الأمراض
الاورام
الامراض الوراثية
الامراض المناعية
الامراض المدارية
اضطرابات الدورة الدموية
مواضيع عامة في علم الامراض
الحشرات
التقانة الإحيائية
مواضيع عامة في التقانة الإحيائية
التقنية الحيوية المكروبية
التقنية الحيوية والميكروبات
الفعاليات الحيوية
وراثة الاحياء المجهرية
تصنيف الاحياء المجهرية
الاحياء المجهرية في الطبيعة
أيض الاجهاد
التقنية الحيوية والبيئة
التقنية الحيوية والطب
التقنية الحيوية والزراعة
التقنية الحيوية والصناعة
التقنية الحيوية والطاقة
البحار والطحالب الصغيرة
عزل البروتين
هندسة الجينات
التقنية الحياتية النانوية
مفاهيم التقنية الحيوية النانوية
التراكيب النانوية والمجاهر المستخدمة في رؤيتها
تصنيع وتخليق المواد النانوية
تطبيقات التقنية النانوية والحيوية النانوية
الرقائق والمتحسسات الحيوية
المصفوفات المجهرية وحاسوب الدنا
اللقاحات
البيئة والتلوث
علم الأجنة
اعضاء التكاثر وتشكل الاعراس
الاخصاب
التشطر
العصيبة وتشكل الجسيدات
تشكل اللواحق الجنينية
تكون المعيدة وظهور الطبقات الجنينية
مقدمة لعلم الاجنة
الأحياء الجزيئي
مواضيع عامة في الاحياء الجزيئي
علم وظائف الأعضاء
الغدد
مواضيع عامة في الغدد
الغدد الصم و هرموناتها
الجسم تحت السريري
الغدة النخامية
الغدة الكظرية
الغدة التناسلية
الغدة الدرقية والجار الدرقية
الغدة البنكرياسية
الغدة الصنوبرية
مواضيع عامة في علم وظائف الاعضاء
الخلية الحيوانية
الجهاز العصبي
أعضاء الحس
الجهاز العضلي
السوائل الجسمية
الجهاز الدوري والليمف
الجهاز التنفسي
الجهاز الهضمي
الجهاز البولي
المضادات الحيوية
مواضيع عامة في المضادات الحيوية
مضادات البكتيريا
مضادات الفطريات
مضادات الطفيليات
مضادات الفايروسات
علم الخلية
الوراثة
الأحياء العامة
المناعة
التحليلات المرضية
الكيمياء الحيوية
مواضيع متنوعة أخرى
الانزيمات
Water Potential
المؤلف:
AN INTRODUCTION TO PLANT BIOLOGY-1998
المصدر:
JAMES D. MAUSETH
الجزء والصفحة:
1-11-2016
5454
Water Potential
Like any other chemical, water has free energy, a capacity to do work. For most chemicals this energy is called its chemical potential. Because water is so important in botany, its chemical potential is usually referred to as water potential and has the symboly (pronounced "sigh"). Water potential, the free energy of water, can be increased several ways Water can be heated, put under pressure, or elevated. The energy of water can be decreased by cooling it, reducing pressure on it, or lowering it.
These examples of change in water's capacity to do work are easy to understand, but it can be changed in other ways as well. When water adheres to a substance, these water molecules form hydrogen bonds to the material and are not as free to diffuse as are other water molecules; their capacity to do work has decreased. Consider a small beaker of water; if it is pure water, it can flow, move, dissolve material, and hydrate substances. But if a sponge is added, water molecules adhere to the sponge material and can no longer flow or easily dissolve things. If a large amount of sugar is added instead of a sponge, the results are the same. Syrup is just a sugar solution, but the water molecules in syrup have less capacity to do work than do the molecules of pure water.
Water potential has three components:
In this equation, yp is the pressure potential, the effect that pressure has on water potential. If water is under pressure, the pressure potential increases and so does water potential. If pressure decreases, so do the pressure potential and water potential. Pressure can be positive (when something is compressed) or negative (when something is stretched). Most liquids cannot be stretched very much, but because water is cohesive, it actually can resist considerable tension. When water is under tension, pressure potentialis a negative number. Potential is measured in units of pressure, usually in megapascal (MPa) or bars. One megapascal is approximately equal to 10 bars or 10 atmospheres of pressure. Pure water at one atmosphere of pressure is defined by convention as having a water potential of zero.
yp is the osmotic potential, the effect that solutes have on water potential. In pure water, no solutes are present and osmotic potential is given the value of 0.0 MPa. adding solutes can only decrease water's free energy because water molecules interact with site molecules and cannot diffuse easily. Therefore, osmotic potential is always negative. If water molecules do not interact with the added molecules, the substance does not dissolve.
It is important to be careful here: Adding acid to water only seems to make water more active. The solution may have more free energy than the water, but it does not have more free energy than both pure water and the original concentrated acid.
Osmotic potential is related to the number of particles present in solution; that is, a solution composed of 2g of glucose in 100 mL of water has an osmotic potential twice as negative as a solution of only 1 g of glucose per 100 mL of water. This has some unexpected results: If a molecule of starch containing 1000 molecules of glucose is hydrolyzed to 1000 free glucose molecules, the osmotic potential of the solution becomes much more negative because there are now 999 more particles in solution than previously. Using terms such as "increase," "decrease," "larger," and "smaller" can be confusing when dealing with negative numbers. If the osmotic potential goes from —0.01 to —0.1 MPa, is it increasing or decreasing? It is least confusing to use the terms "more negative," "less positive," and so on.
ym is the matric potential, water's adhesion to non-dissolved structures such as cell walls, membranes, and soil particles. Adhesion can only decrease water's free energy, so matric potential is always negative. In soils, matric potential is important because so much of the soil water is tightly bound to soil particles. But in living cells, matric potential usually is much less important than osmotic potential or pressure potential and usually is ignored entirely (Table 12.1).
FIGURE 1: (a) The material on the left is potassium hydroxide; that on the right is starch. They were photographed immediately after being exposed to air, while they were dry. (b) Photographed after 1 hour in humid air. Water has moved from where it was more concentrated (the air) to less concentrated. The potassium hydroxide holds water by forming a solution with a very negative osmotic potential. Water is held to the starch by adhering to the long polysaccharide molecules. Water is not obvious in the starch, but think of saltine crackers left unwrapped. (c) By adding salt to eggplant, water can be drawn from the tissues, making them easier to cook.
The movement of water is related to water potential; substances diffuse from regions where they are more concentrated to regions where they are more dilute. This can be stated more precisely for water: Water moves from regions where water potential is relatively positive to regions where water potential is relatively more negative (Fig. 1). This statement contains several important points:
1. Water moves whenever there is a difference in water potential within the mass of water. All protoplasts are interconnected and most cell walls are fully hydrated, so basically all of a plant body is one mass of water; water can move between regions in the plant if the water potentials of the regions are not equal (Fig. 3a). As a consequence, the water potential of any particular cell may change many times a day as various parts of a plant lose or gain moisture (Fig. 3).
FIGURE 2:(a) If a solution whose water potential is —0.1 MPa is connected to a solution whose water potential is —0.2 MPa, the two are not in equilibrium. Water moves from a region of relatively positive water potential to a region of more negative potential, as indicated. Water molecules move in both directions, but more move to the right in any particular instant. Both beakers must have solute dissolved in them (otherwise each would have y = 0.0 MPa), and the -0.2 MPa solution must have twice as much solute. Because the concentration of solute molecules in the left beaker is lower, they are less able to restrict the movement of water molecules than those in the right beaker, where the greater concentration of solute molecules is more able to restrict water movement. (b) If the water potential of two solutions is identical, they are in equilibrium and no net movement of water occurs; in each second, equal numbers of water molecules move to the right and left. (c) A y of —1.0 MPa is very negative and has a strong tendency to absorb water, but we cannot be certain that water will move to it in this case; the right beaker may contain a solution with a y of -1.1 MPa.
the plant if the water potentials of the regions are not equal (Fig. 2a). As a consequence, the water potential of any particular cell may change many times a day as various parts of a plant lose or gain moisture (Fig. 3).
2. If the water potentials of two regions are equal, the regions are in equilibrium and there is no net movement of water. Water still diffuses back and forth, but, on
average, equal numbers of water molecules diffuse into and out of a site (Fig. 2b).
3. Water potentials must always be considered in pairs or groups. Because water moves from one site to another, the water potentials of the two sites are important. Knowing one single water potential does not allow us to predict whether water will move (Fig. 2c).
Femperature is not a factor, because the solutions being compared are assumed to be at the same temperature. This is not strictly correct if the water potentials of leaves and roots are compared, but the difference is not significant.
FIGURE 3:If soil is watered well and then allowed to dry over a period of days, the water potential of the soil solution gradually and smoothly becomes more negative. Every day, leaf transpiration loses water more rapidly than xylem transport replaces it, so leaves dry slightly and leaf water potential becomes more negative. At night, stomata close and xylem transport rehydrates the leaf tissue, but in each cycle, the leaves dry more than the previous night. This is not very serious until daytime leaf water potential becomes more negative than the wilting point (dashed line). When leaves wilt, many metabolic processes are adversely affected. Wilting point varies from species to species and is much more negative for xerophytes than for mesic plants. Once soil becomes extremely dry, even nighttime rehydration does not bring leaf water potential above the wilting point; the plant is at its permanent wilting point and severe stress damage may occur. All growth stops, and leaves and developing flower buds or fruits may die and be abscised.
CELLS AND WATER MOVEMENT
Some examples may help to explain the importance of water potential. Imagine a cell with a water potential of —0.1 MPa. It contains solutes that cause the osmotic potential to be some unknown negative number. The cell is turgid and presses against the cell wall, but the cell wall presses back equally, causing pressure on the cell, and the pressure potential is some unknown positive number. We can ignore matric potential because it is usually such a small number. The osmotic potential, whatever it is, plus the pressure potential, whatever it is, equals —0.1 MPa (Fig. 4a). Now imagine the cell being placed in a beaker of solution that also has a water potential of —0.1 MPa. The two water potentials are equal, the cell and solution are in equilibrium, and no net water movement occurs—the cell neither shrinks nor swells. Water molecules do move between the cell and the solution, but approximately equal numbers move in each direction every second (Fig. 4b).
FIGURE 4 (a) A cell whose y is -0.1 MPa but whose values of yp or yp are unknown. (b) If the cell is placed in a solution with a y of —0.1 MPa, the cell is in equilibrium with the solution and no net movement of water occurs. (c) If the cell is placed in a solution with a y of —0.3 MPa, the cell loses water to the solution until the cell's water potential is also —0.3 MPa (d), even if the cell is killed by the loss of water. The fact that the cell's water potential becomes more negative means that the osmotic potential or the pressure potential or both also become more negative.
Now imagine the same cell placed in a solution with a water potential of —0.3 MPa (Fig. 4c). The water potentials are not equal, that of the solution being more negative (has more solutes) than that of the cell, so water moves from the cell into the solution. How much water moves? As water leaves the cell, the solutes that remain in the protoplasm become more concentrated. Because more solutes are present per unit water, osmotic potential becomes more negative. As water moves out, the protoplasm volume becomes smaller, the protoplast presses against the wall with less force and the wall presses back less, so pressure potential becomes less positive. Because both osmotic potential and pressure potential are decreasing (becoming more negative), so is the water potential of the cell. At some point the cell's water potential (y cell) reaches —0.3 MPa and is in equilibrium with the solution; then net water movement ceases (Fig. 4d). Of course, as water moves from beaker, the solution becomes more dilute and its osmotic potential and water potential become less negative; its pressure potential does not change because pressure cannot build up in an open beaker. Therefore, equilibrium actually occurs slightly above —0.3 MPa; but because most beakers are much larger than most cells, the amount of water that moves is much more significant to the cell than to the beaker solution.
Consider the relative importance of osmotic potential and pressure potential in this example. In order for osmotic potential to become twice as negative (for example, from —0.15 MPa to —0.3 MPa, the cell has to lose half its water or double the number of solute particles. Either action is drastic; almost any cell dies if it loses half its water, so osmotic potential does not usually increase or decrease more than a few megapascals. Pressure potential can change enormously, however, usually with movements of only small amounts of water. Consider the top of a table: Its molecules are pressing upward with exactly the same force that gravity is pulling them downward. Placing a book on the table causes the table's molecules to exert more pressure upward as their bonding orbttals are stretched (Fig. 5). The table changes the amount of upward pressure it can exert with very little change in shape.
FIGURE 5 (a) The molecules in this table top are exerting just enough pressure upward to counteract the gravitational force. We know this because there is no movement upward or downward of the table top, so forces must be in equilibrium. (b) Adding several books increases the gravitational force, but no net movement occurs. The table top is slightly bent, which stretches its molecules, but they resist just enough to counter the new force. (c) Even more force is perfectly balanced by the table top. If the books were removed, the stretching on the table would stop and the molecules would go back to exerting only enough pressure to counteract its own weight—the table would not fly upward.
A similar process occurs in cell walls; imagine placing the cell, now with a water potential of —0.3 MPa, in a beaker of pure water. Some water moves inward, diluting the solutes and causing the osmotic potential to become less negative, but the change is not significant. However, the extra volume of the water causes the protoplast to swell and press against the wall with more force. The wall presses back with equal force and pressure potential rises rapidly, even though only a small amount of water moves in (Fig. 6). How high will it rise? We cannot predict its value, but it will go high enough that osmotic potential plus pressure potential will equal zero, and the cell will be in equilibrium with pure water.
FIGURE 6: (a) A healthy cell, turgid and full of water and protoplasm; it is swollen and firm, just like the cells of unwilted leaves. Its walls are stretched and are pressing back against the protoplasm that is pressing on them. (b) If even a small amount of water enters the cell, the slight increase in dime causes the walls to stretch even more, but they push back, and pressure builds inside the cell. Consequently, the yp, becomes much more positive and so does the water potential of the cell. Because of the slight increase in the amount of water in the cell, the salts, sugars, amino acids, and all other solutes are now slightly more dilute, so yp becomes slightly less negative also, but this change is insignificant compared with the change in yp.
Can a cell ever absorb so much water that it bursts? Animal cells often burst if placed in pure water, a process called lysis, but plant cells can never burst (Fig. 7). Walls, either primary or secondary, are always strong enough to resist breakage by water absorption. Even the thinnest, most delicate walls of mature parenchyma cells can exert enough pressure on the protoplast to raise the pressure potential high enough to counterbalance osmotic potential, however negative it might be.
FIGURE 7:Some aspects of biotechnology processes require that the cell wall be digested away with cellulase enzymes, leaving behind a naked protoplast. The digestion mixture must contain just enough solute—usually sucrose or the sugar-alcohol mannitol—that the cells lose water and shrink away from the wall. If not, the protoplasts burst when the wall is removed. The solute concentration must be adjusted carefully; if it is too strong, too much water is pulled out of the cells and they die. (a) Cells in suspension culture before treatment. (b) Cells in 6% mannitol with the protoplasts just pulling back from the wall (both X 500).
Immature, growing cells have weak, deformable walls and cannot generate enough pressure to stop water absorption. The cell grows rather than bursts. Under these conditions, the cell may increase greatly in size. With such a large influx of water, solutes in the cell may become significantly diluted, osmotic potential and water potential may become less negative, and the cell reaches hydraulic equilibrium with surrounding cells and growth stops. However, in growing regions, such as the tips of stems and roots, and in expanding leaves, cells can keep their osmotic potential and water potential very negative despite the influx of water, either by actively pumping in solutes through the plasma membrane or by hydrolyzing giant starch molecules into thousands of glucose molecules. Once the proper size is reached, growth can be stopped either by strengthening the wall so that it exerts more pressure and raises the pressure potential or by stopping the import of solutes or the hydrolysis of starch, allowing the osmotic potential to rise. Either way, rising pressure potential or osmotic potential causes the cell's water potential to rise and reach equilibrium with the surrounding cells, stopping the net inflow of water.
Although plant cells cannot absorb so much water that they burst, water loss can be a serious problem. Imagine that our demonstration cell, now in pure water and with a water potential of 0.0 MPa, is placed in a strong sugar solution with a water potential of -2.0 MPa. Water moves out of the cell, osmotic potential becomes slightly more negative, and the pressure potential drops rapidly. In such a strong solution, long before the cell reaches equilibrium it loses so much water that the protoplast shrinks in volume and no longer presses against the wall. The wall is not stretched and does not exert any pressure back, so the pressure potential drops to 0.0 MPa. The point at which the protoplast has lost just enough water to pull slightly away from the wall is called incipient plasmolysis and is quite important (Figs. 8 and 9). Up to that point the cell has lost very little water, so its volume change and osmotic potential change have not been great. But because the pressure potential is now zero, the water potential equation is
If the cell has not reached equilibrium at the point of incipient plasmolysis, it continues to lose water and the protoplast pulls completely away from the wall and shrinks. The ceil has become plasmolyzed. Water potential continues to become more negative entirely owing to the osmotic potential as the solutes become more concentrated. Most plants at the equilibrium point of — 2.0 MPa would die of severe water loss.
FIGURE 8: (a) When placed in a solution with a strongly negative water potential, the cell loses water rapidly and cell volume drops. yp becomes less positive and ycell becomes more negative; yp changes criy a small amount. (b) Incipient plasmolysis is the point at which the protoplast has shrunk just enough to pull away from the wall, so yp is zero and ycell equals yp. (c) If the cell does not reach equilibrium at incipient plasmolysis, it continues to lose water, and ycell continues to become more negative until it reaches — 2.0 MPa. The pressure potential here cannot become a negative number, so the changing water potential is due to a changing osmotic potential. During plasmolysis, the cell loses enough water to change the concentration of solutes significantly.
FIGURE 12.12: (a) These cultured cells have been placed in 12% mannitol for several hours and are severely plasmolyzed (compare with Fig. 12.10). (b) After a few days of severe plasmolysis, the cells have died (both X 500).
Although such severe desiccation kills most cells, some can survive it easily. The embryos in most seeds are much drier, having water potentials as low as — 20 MPa. Less dramatically, the leaves of desert shrubs in dry soil have water potentials as low as — 2.0 to
- 6.0 MPa (Table 12.2). For most plants of temperate climates, a leaf water potential below
- 1.0 MPa stops leaf growth, although leaves can survive such desiccation for many days or weeks.
SHORT DISTANCE INTERCELLULAR TRANSPORT
Most plant cells communicate with their neighboring cells, transferring water, sugars, minerals, and hormones at least. This movement occurs by a variety of mechanisms. First, all living cells are interconnected by plasmodesmata, the fine cytoplasmic channels that pass through primary cell walls. All the protoplasm of one plant can be considered one continuous mass, referred to as the symplast.
Material also is transferred from one cell to another by transport across the plasma membrane. The methods involve osmosis, molecular pumps in the plasma membrane itself, or fusion between transport vesicles and the plasma membrane. Once across a plasma membrane, a molecule initially resides in the cell wall. The wall is probably thin and permeable, and the molecule can penetrate it easily, diffusing across it to an intercellular space or laterally through it, spreading along the cell surface (Fig. 10). Most small molecules can move easily through both the wall and the intercellular spaces; the two together are called the apoplast of the plant.
FIGURE 10 (a) Once released from a cell, a molecule diffuses in a series of random short paths as it collides with and bounces off other molecules. Probability favors its entering a neighboring cell (molecules 1,2, and 3), but it can diffuse laterally along the wall as well (molecules 4 and 5). (b) In many glands, the apoplast is large, so movement between cells may be faster and easier than movement within cells. Such glands often have a lining of compact tissue that isolates the gland, preventing the secreted material from permeating the whole region.
In glands, the apoplast is mostly intercellular space through which molecules move easily, usually toward the surface of the gland. In non-glandular regions, the apoplast is mostly cell wall. The secreted molecule is probably absorbed by a cell neighboring the one that secreted it. In most parenchymatous tissues, primary walls are thin (less than 1 pm thick), and the contact faces between two cells are so extensive (10 to 20 pm2) that the probability is much greater that a molecule will either diffuse more or less directly into the next cell or re-enter the cell from which it came (Fig. 11). If the molecule was originally secreted by active transport, the original cell membrane is probably impermeable to it, at least in that area, so return to the original cell is usually not possible. This is probably the most common mechanism for the movement of water, sugar, and other nutrients between parenchyma cells within the cortex, pith, or leaf mesophyll.
FIGURE 11:Primary walls are usually so thin that few molecules can diffuse sideways; most move rather quickly into the next cell. Washingtonia filifera seed epithelium (X 4,700). (Courtesy of Dadeen A. De Mason, University of California, Riverside)
GUARD CELLS
The opening and closing of stomatal pores are based on short distance intercellular transport. At night, when stomata are closed (except for CAM plants), guard cells are somewhat shrunken and have little internal pressure. Water can enter or leave them easily, because water crosses any biological membrane rather freely. But the guard cells are in hydraulic equilibrium with surrounding cells: Water enters and leaves guard cells at approximately the same rate, and no net change occurs in the amount of water. When guard cells must open, such as just after sunrise, potassium ions (K+) are actively transported from surrounding cells into guard cells (Fig. 12). Once inside the guard cells, the potassium cannot leave because the plasma membrane is impermeable to it: Potassium pumping is possible but diffusion is not. The loss of potassium causes the water potential in adjacent cells to become less negative, whereas absorption of potassium causes the water potential in guard cells to become more negative. Adjacent cells and guard cells are thrown out of hydraulic equilibrium by the pumping of potassium ions, and water diffuses out of surrounding cells across their plasma membrane, across the two primary walls and middle lamella, and into guard cells across their plasma membrane. The extra water and potassium cause guard cells to swell, bend, and push apart, opening the stomatal pore. Once they are open, potassium pumping stops, water movement brings guard cells and adjacent cells into water potential equilibrium again, and net water movement stops. When guard cells must close, the process is reversed: Potassium is pumped from guard cells into surrounding cells and water follows. Notice that guard cells and adjacent cells are in equilibrium when stomata are fully open and fully closed.
Notice also that guard cells of fully opened and fully closed stomata are both in equilibrium with surrounding cells (and thus also with each other), even though they all have different internal conditions: When open, the guard cells' abundant potassium gives them a very negative osmotic potential which is countered by turgor pressure, giving a large pressure potential. This results in only a small negative water potential. When closed, the guard cells have less potassium, so only a small negative osmotic potential; this is countered by less turgor, and therefore a less positive pressure potential. These cells too have only a small negative water potential. Even though two cells might have very different internal conditions, they can have the same water potential and be in hydraulic equilibrium.
FIGURE 12:At night, guard cells and adjacent cells are in hydraulic equilibrium (a), but at sunrise, potassium is pumped into the guard cells, increasing solute concentration (b). Osmotic potential and water potential become more negative and water flows in (c), causing the guard cells to swell and open the pore. As pressure builds, pressure potential rises, counteracting the falling osmotic potential, so the guard cell's water potential rises and moves back into equilibrium with the adjacent cell, but by the time that happens, the stomatal pore is open (d). At night, when the stomata must close, all steps are reversed.
MOTOR CELLS
The leaves of sensitive plant (Mimosa pudica), prayer plant (Oxalis), and many other species are able to move slowly and reorient themselves by flexing and folding in response to a variety of stimuli (Fig. 13). The location of flexure is either the entire midrib or the point at which the petiole attaches to the lamina or stem. The cells at these "joints," called motor cells, are similar to guard cells: They can either accumulate or expel potassium and thus adjust their water potential and turgidity.
In Venus' flytrap, the leaf can close rapidly, in less than a second, but it requires several hours to reopen. Motor cells are located along the midrib, and when they are shrunken, pressure in other midrib cells causes the two halves of the blade to be appressed, and the trap is closed. Trap opening occurs as potassium is slowly accumulated by motor cells, water diffuses in, and the motor cells become turgid. Closure is not caused by pumping potassium out of the motor cells; that would be too slow to be effective. Instead, the membrane suddenly becomes freely permeable to potassium and it rushes out instantly. The water balance is rapidly changed and water too floods out, allowing the motor cells to virtually collapse, and the trap shuts quickly enough to catch insects.
FIGURE 13: (a) Each leaflet of the compound leaf of Oxalis is joined to the petiole by motor cells; here they were photographed in the morning when it was cool and the sunlight was not intense. Motor cells are turgid and leaflets are held into the sunlight, (b) Oxalis plants in full, intense sunlight. The motor cells have lost potassium and water; thus they are not turgid. As a result, the leaflets hang down, minimizing their exposure to light. Later in the afternoon, when sunlight is not so intense, or if the shadow of a tree moves across the plant, the motor cells will absorb potassium, then water, and raise the leaflets again.
TRANSFER CELLS
The rate at which material can be actively transported depends on the number of molecular pumps present, which in turn depends on the surface area of the plasma membrane: The larger the membrane, the more molecular pumps it can hold. In certain specialized transfer cells, the walls are smooth on the outer surface but have numerous finger-like and ridge-like outgrowths on the inner surface. The plasma membrane is pressed firmly against all the convolutions and thus has a much larger surface area than it would if the wall were flat. Consequently, room is available for many molecular pumps, and high-volume transport can occur across these transfer walls. Transfer cells are found in areas where rapid short distance transport is expected to occur: in glands that secrete salt, in areas that pass nutrients to embryos, and in regions where sugar is loaded into or out of phloem.
الاكثر قراءة في مواضيع عامة في علم النبات
اخر الاخبار
اخبار العتبة العباسية المقدسة
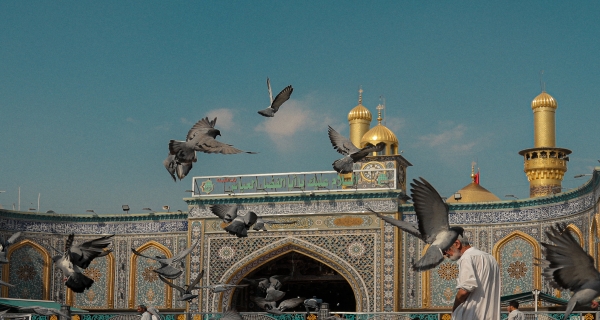
الآخبار الصحية
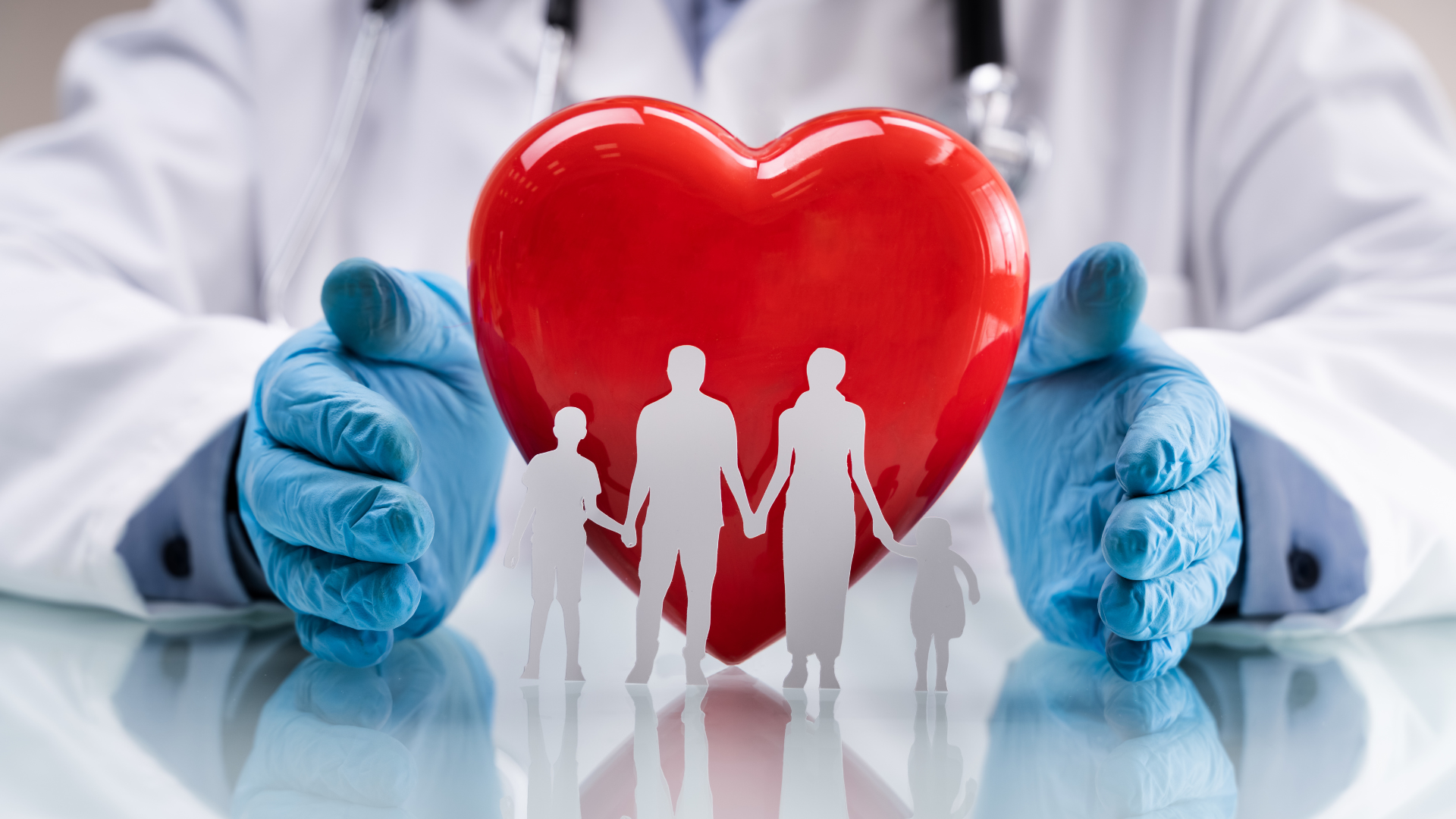